SALTS IN THE DETERIORATION OF POROUS MATERIALS: AN OVERVIEW
A. ELENA CHAROLA
ABSTRACT—ABSTRACT—The present overview on salt-induced deterioration of inorganic porous materials focuses on those topics considered the most relevant for understanding this phenomenon. These are movement of both water/moisture and salts within the pore system; influence of the presence of salts in moisture absorption; effect of the simultaneous presence of more than one salt; salt distribution in masonry; deterioration mechanisms; and weathering patterns. Of the latter, the most ubiquitous are powdering and scaling, and they have been found to occur on most stone types. However, the mechanism that induces the same outward appearance on different stones can vary significantly depending on the synergism that develops among porous material, salt, and moisture.
TITRE—La détérioration des matériaux poreux par les sels: une vue d'ensemble de la question. RÉSUMÉ—Cet article présente un tour d'horizon du phénomène de la détérioration des matériaux inorganiques poreux causée par les sels. Il met l'accent sur ce que l'on considère être les facteurs les plus importants pour expliquer le phénomène: le déplacement de l'eau ou de l'humidité, ainsi que des sels à l'intérieur des pores; l'effet causé par la présence des sels sur l'absorption de l'humidité; l'effet de la présence simultanée de plus d'un type de sel; la distribution des sels dans la maçonnerie; les mécanismes de la détérioration; les effets du vieillissement naturel. Parmi ces derniers, l'effritement et l'écaillage sont ceux que l'on rencontre le plus souvent et la plupart des types de pierre peuvent en être victimes. Cependant, bien que la détérioration de divers types de pierres puisse sembler la même en surface, le mécanisme qui cause l'altération peut varier grandement en fonction du synergisme qui se développe entre le matériau poreux, le sel et l'humidité.
TITULO—Una reseña sobre la presencia de sales en el deterioro de materiales porosos. RESUMEN—La presente reseña puntualiza los tópicos más importantes que aclaran el proceso de deterioro de materiales inorgánicos porosos por el efecto de la presencia de sales. Estos tópicos son: movimiento del agua/humedad y sales dentro del sistema de poros; influencia de la presencia de sales en la absorción de humedad; efecto de la presencia simultánea de dos o más sales; distribución de las sales en mampostería; mecanismos de deterioro y tipos de deterioro (weathering patterns). De estos últimos, los más comunes son la pulverización y la delaminación (o escamación) que pueden ocurrir en casi todos los tipos de rocas. Sin embargo, aunque el fenómeno es aparentemente idéntico, el mecanismo que lo produce puede variar significativamente dependiendo del sinergismo generado entre el material poroso, la sal y la humedad.
“I observed … that salt exuded from the soil to such an extent as even to injure the pyramids.”
—Herodotus, (484 B.C.–425 B.C.), History, bk. 2
2 1. INTRODUCTION
It has long been known that inorganic porous materials, such as rocks and masonry materials, are susceptible to deterioration when salts, in particular highly soluble salts or hydrate-forming salts, are found within them. J. Iñiguez Herrero (1967) gives an excellent overview of the earliest papers, published around 1910, that clearly relate the presence of soluble salts to the deterioration of stone. Some years later another, more comprehensive review was published by I. Evans (1969–70). A. Goudie and H. Viles (1997) emphasize the influence that geomorphologists, notably H. Mortensen, had in developing the field of salt weathering.
In his ground-breaking paper, Mortensen (1933) contested the then-accepted view that the damage observed on rocks in the desert was caused by the crystallization of salts, a phenomenon compared to that of freezing water. He argued, instead, that crystals precipitating out of a saturated solution by evaporation occupy less volume than the original solution, and, therefore, no crystallization pressure can develop. He attributed deterioration to pressure developed upon volume increase when a salt hydrates. To illustrate the point, he advanced an equation to calculate hydration pressures for calcium sulfate, sodium sulfate, magnesium sulfate and sodium carbonate, typically salts found in desert areas. This theoretical approach was complemented by the work of C. Correns and W. Steinborn (1939), who studied both crystallization and hydration pressures in detail. However, as W. Duttlinger and D. Knöfel (1993) pointed out, few and inconclusive attempts have been made to validate the hydration pressure theory experimentally. The last authors also give an excellent review of the literature on salt crystallization and theory.
Although there is a vast literature on this complex subject, or maybe because of it, the deterioration of materials is often simplistically attributed to “the presence of salts” or, at best, to the crystallization—or hydration—pressure of a particular salt. The present overview aims to present a coherent picture of the many mechanisms that are involved in the deterioration of porous materials by salts. For damage to occur, salts must move into and within porous bodies, a process that requires the presence of water (liquid) and/or moisture (water vapor). Thus, understanding how water moves is fundamental to elucidating the deterioration mechanism(s) that salts may have on porous materials.
3 2. ORIGIN OF SALTS
As C. Price (1996) summarizes in his review on stone deterioration, salts can originate from various sources: air pollution, deicing salts, soil, sea spray, inappropriate treatments, or interaction between building materials. Some materials may even contain salts inherently. For example, Portland cement usually contains alkali sulfates that are released from concrete or cement mortars; and bricks, if not fired adequately, may contain sodium sulfate. This salt becomes evident as efflorescences on random bricks soon after construction of the masonry. Historic buildings, over centuries, may accumulate a high concentration of salts, particularly if some sections of them had been used for salt storage or as stables.
4 3. MOVEMENT OF SALTS IN POROUS BODIES
Salts can enter and move through porous bodies only when dissolved in water. Therefore, understanding the movement of this fluid in the material in question is of prime importance.
4.1 3.1 WATER MOVEMENT
Water can enter a porous material either as liquid or vapor. In the liquid state, two mechanisms can be operative: capillarity and/or infiltration. While the first is a result of the attraction of the water and the capillary material as well as the surface tension of the liquid, the latter requires a hydrostatic pressure and depends on the permeability of the material. Both mechanisms have been thoroughly studied and clearly described in standard texts (Feilden 1982; Amoroso and Fassina 1983; Weber 1984; Massari and Massari 1993; Henriques 1994).
In the vapor state, water can enter a porous material through two main mechanisms: condensation and hygroscopicity. Two types of condensation should be distinguished: surface condensation and microcondensation (or capillary condensation) in pores. Both are clearly understood and differentiated (Camuffo 1998). Hygroscopicity, on the other hand, is a broad term used to cover different processes for “absorbing or attracting moisture from the air” as defined by the American College Dictionary (1962). First of all, the material itself can sorb—i.e., ad- and/or ab-sorb—a certain degree of moisture depending on its nature, porosity, and internal surface. Second, salts can also absorb moisture, especially when the relative humidity increases above their equilibrium RH. Then, particularly the very soluble ones may deliquesce—i.e., absorb so much water vapor that they form a saturated solution. The term “deliquescent” is preferable to “hygroscopic” to describe this phenomenon because it identifies the condition of “becoming liquid.” Finally, concentrated salt solutions have a lower vapor pressure than pure water and have a higher tendency to condense water vapor from the environment to reach equilibrium—i.e., to equalize the activity of water within the solution to that in the vapor phase. This last process has also been called “classical osmosis,” particularly in German texts (Weber 1984, 29).
It is important to understand how water will move once inside a porous material. If it moves as a liquid, it will be able to transport salts; if as a vapor, it may be retained through hygroscopicity. In the first case, the mechanism relies on capillarity, and, in the second, on diffusion. The transition point between these two mechanisms defines the critical moisture content, Ψc, of a porous material. This parameter is a constant for each material and depends mainly on porosity and pore-size distribution (Snethlage and Wendler 1997).
The actual distribution of moisture within stone depends on porosity, pore-size distribution, and environmental conditions. As R. Snethlage and E. Wendler (1997) discuss, the maximum of moisture content resulting from wet-dry cycling is closer to the surface in denser stones, and deeper and broader in coarse, porous materials.
Lastly, it should be remembered that when porous materials absorb moisture or liquid water, they expand. This effect is known as hygric dilatation, if moisture is absorbed, or hydric dilatation, when it is produced by liquid water. When the material dries, it will shrink. Upon subjection to wet-dry, or moist-dry cycling, the resulting expansion and contraction can induce material fatigue (Snethlage and Wendler 1997). This effect can be compounded or inverted when salts are present, as discussed in section 7.
4.2 3.2 SALT MOVEMENT
Salts within a porous material will increase the amount of water in it, partly by enhancing capillary rise, as suggested by G. Massari and I. Massari (1993), but also because of hygroscopicity (Weber 1984). This is a particularly important point when decisions have to be made for moisture mitigation in a building. If moisture in a wall is caused mainly by high salt content, an expensive damp-proof barrier will be useless in solving the humidity problem.
Two main mechanisms are responsible for the introduction of soluble salts into the porous material of a building: capillary rise of groundwater and infiltration by rainwater. The former is responsible for introducing soil or deicing salts, while the latter will contribute salts resulting from air pollution or marine aerosols (Behlen et al. 1997). Both these processes rely on capillary transport of water into the pore system as described by B. Vos (1971) and, in more detail, by D. Camuffo (1998). A third mechanism that can introduce, or reintroduce, surface salts into the materials is surface condensation. Camuffo (1998) addresses this issue thoroughly.
Once a salt is in a porous material, its movement will be strongly dependent on ambient conditions—i.e., temperature and relative humidity—as well as the presence of other salts. Changes in relative humidity result in its partial crystallization and dissolution. These phenomena take place preferentially in medium to large pores and result in movement of solution from smaller pores to the larger pores (Camuffo 1998). From actual field observations, it has been established that salt crystals form mainly in pores 1–10 µm in diameter (Zehnder and Arnold 1989). The presence of a second salt results in the lowering of the relative humidity required for precipitation (Price and Brimblecombe 1994; Steiger and Dannecker 1995), thereby increasing the frequency of the crystallization-dissolution cycling.
Creep—i.e., salts crystallizing on glass walls above the surface of a solution—has also been postulated as a model for the mechanism of salt movement within a porous structure (Pühringer 1983a). This phenomenon can be readily observed for solution films drying out on glass surfaces. Salts crystallize at the edge of the film, forming a fine-grained microporous salt body. This enhances the capillary flow toward the edge of the film, advancing the crystallizing front. As J. Pühringer (1983b) graphically states, the salt carries the water.
5 4. SALT SOLUTIONS
As is known, the water vapor pressure over a salt solution is lower than the vapor pressure of pure water at a given temperature. As the concentration of the solution increases, the water vapor pressure decreases, reaching its lowest value when the solution is saturated. This water vapor pressure, which depends on the nature of the salt in the solution and temperature, corresponds to what is known as the “equilibrium relative humidity” since water vapor pressure can be expressed as %RH. For example, at 20°C, the values can range from 99% for calcium sulfate dihydrate (gypsum) to 11% for lithium chloride monohydrate, depending on the interaction of the particular ions with the water molecules.
Seldom can the deterioration of porous materials be attributed to the presence of only one salt. In general, two or more salts are found to be present. The simultaneous presence of two salts affects their behavior in solution and, in particular, their individual solubility. The rule of thumb is that if the salts do not have any ions in common, as in the case of a mixture of sodium chloride and calcium sulfate dihydrate (gypsum), solubility of both salts increases because of the higher ionic strength of the solution. However, the increase in solubility will be far greater for the less-soluble salt, in this case gypsum. If the salts have an ion in common, such as sodium chloride or sodium sulfate, solubility of both will decrease. Again, the solubility of the less-soluble salt, sodium sulfate, will be affected more than that of the more soluble one. However, not all salts behave in this way; for example, the solubility of potassium nitrate increases in the presence of other nitrates (Steiger and Dannecker 1995).
A solution of a mixture of salts will not have a single “equilibrium RH” at a given temperature but will show a range of such humidities (Price and Brimblecombe 1994). This range will not necessarily fall between the levels of the equilibrium RH of the individual salts and can even be broader, as is the case for the mixture of potassium chloride and sodium chloride: the equilibrium RH ranges from close to 85% to 72.4%, while equilibrium RH for the individual salts is 84.3% and 75.3%, respectively (Steiger and Dannecker 1995). Further details about the model used to calculate the behavior of multiple salt systems can be found in C. Price and P. Brimblecombe (1994), M. Steiger and A. Zeunert (1996), and Steiger et al. (1998). The effect of a wider RH range of a mixed solution within the pore system of a material will be compounded by the hygroscopicity and capillary condensation capability of the latter, increasing the deterioration potential of the salt(s).
Also to be taken into account is the order in which salts will precipitate out of a multiple salt solution. This order depends on the composition of the original mixture present and has been studied by geologists for the case of evaporating lakes. Hence, mixtures of these soluble salts are referred to as “evaporites.” The order of precipitation of such mixtures can be found in most geochemistry textbooks (Krauskopf 1979) or in A. Goudie and H. Viles (1997).
6 5. SALT DISTRIBUTION IN MASONRY
On a wall with rising damp, salts crystallize at different heights depending on their solubility. A. Arnold (1982) has synthesized this distribution from his careful analysis and observation of many damp structures. He differentiates four zones: the first (A), close to the ground, shows less damage than the second (B), which in general is the most deteriorated one. Above this zone, the third area (C) is characterized by being darker than the others; it marks the upper limit of moisture rise. The fourth area (D) is sound wall. In area A, the more insoluble salts such as gypsum and the calcium and magnesium carbonates crystallize. In zone B, potassium nitrate and magnesium and sodium sulfates precipitate out, forming efflorescences and crusts. In zone C, the most soluble salts, sodium nitrate and chloride, as well as the deliquescent salts, magnesium nitrate and chloride, are found. The latter will tend to keep the wall moist except under very dry conditions. As Arnold points out, this distribution will be affected if alkaline salts are introduced into the system, as is the case when Portland cement or other alkaline materials are injected to prevent rising damp.
The distribution of salts within a wall is also dependent on the actual mixture of salts present and their origin. As Steiger (1996) discusses, on the basis of the thorough analysis of the north facade of a former convent in northern Bavaria and other monuments (Steiger et al. 1993), the presence of nitrate, potassium, and magnesium, as well as chloride and sodium, is the result of rising damp. The zone of maximum enrichment, around 2–3 m from the ground, reflects the capillary rise height. Fractionation during this ascending transport is revealed by the shift in the potassium/magnesium (K/Mg) ratio, which decreases with increasing height. Sulfate distribution, mainly on the surface of the wall, reflects deposition from the atmosphere. Given normal outdoor environmental conditions, only a small amount of potassium nitrate (niter) can be expected to crystallize, while most other salts, except the rather insoluble gypsum, remain in solution. This analysis confirms the findings of K. Zehnder (1993, 1996), who describes the formation of thin veils of gypsum in the upper zone of the capillary rise. These veils cause a slow decay that is particularly evident on painted stones and wall paintings, where the thin surface layer is especially susceptible to damage.
Steiger et al. (1997) and Behlen et al. (1997) present a careful analysis of the various pathways for sodium chloride accumulation in marine environments. Its distribution within a wall or walls of matched age and material results from the accumulation pathway followed. For example, the significantly higher salt accumulation in one building could be attributed to overall poor design that led to rising damp and increased direct rainwater flow from the roof.
Further studies by Arnold and coworkers (Arnold and Kueng 1985; Arnold and Zehnder 1985) report on efflorescing salts, their distribution over wall surfaces, and their various growth habits. These authors give a good description of the various types of crystals, including longer (fluffy) or shorter (bristly) acicular efflorescences, salt crusts, and salt pustules—i.e., salt crusts limited to small areas. Acicular (whisker) growth occurs at low supersaturation and low evaporation rate. Salt crusts will form in the damper areas of the walls—i.e., in the lower part—and are generally formed by the lesser soluble salts: calcite, gypsum, hydromagnesite, and nesquehonite. But any salt can produce crusts under the right conditions. Arnold and his coworkers also point out that efflorescences age with time and changing conditions due to dissolution and recrystallization processes that may affect the damage induced.
Arnold and Zehnder (1988) also present the results of a five-year monitoring program correlating microclimate and efflorescing salts in several buildings in Switzerland. They relate in situ–measured relative humidity and temperature to the “fractionation” pattern of efflorescing salts as saline solutions rise in walls. Sodium nitrate (nitronatrite or soda niter), for example, was found to effloresce only when the relative humidity dropped below 60% (equilibrium RH for this salt is 75.4% at 20°C). These authors also monitored the aging of the efflorescence as conditions changed. The acicular growth of the nitronatrite, for example, turned into a more isometric shape with time.
If a conservation treatment, such as consolidation or hydrophobization, is applied to salt-containing masonry, the distribution of the salts can be significantly affected. L. Franke and F. Pinsler (1998) proposed an in situ radiography method for measuring the movement of salts resulting from an imperfect surface hydrophobization or when unhydrophobized joints are present. Using salts such as lead nitrate and the hygroscopic sodium iodide for the study they found that salts accumulated at nonhydrophobized surfaces as well as behind the hydrophobized front.
7 6. DETERIORATION MECHANISMS
There is no question as to the damage induced by the presence of salts in porous materials. The problem lies in explaining why and how damage occurs. The earliest postulated theories (Correns 1926; Correns and Steinborn 1939; Correns 1949) regarded crystallization pressure as responsible, and two mechanisms were considered: hydrostatic crystallization pressure and linear crystal growth pressure. Thirty years later, a thermodynamic model was developed for calculating crystallization pressure (Wellman and Wilson 1965). Hydration pressure was first suggested by Mortensen (1933) and has only recently been challenged. These theories and other proposed mechanisms are discussed in the following subsections.
7.1 6.1 CRYSTALLIZATION PRESSURE
Hydrostatic crystallization pressure can develop when a supersaturated solution occupies a smaller volume than the precipitating crystals plus the residual saturated solution, as is the case for most common salts (Correns 1926). Such pressure is directly proportional to the supersaturation ratio (C/Cs)—i.e., the ratio of concentrations of the solute in the supersaturated (C) to the saturated solution (Cs). However, when a pressure develops, the solubility of the salt also increases, according to the Le Chatelier principle, thus diminishing the supersaturation ratio. Therefore, as Duttlinger and Knöfel (1993) point out, this type of mechanism cannot account for the observed deterioration of porous stones by salts.
Linear crystal growth pressure and the equation developed for this mechanism are propounded in a widely quoted English paper by Correns (1949). The equation is:
Equation 1.
 |
Although G. Amoroso and V. Fassina (1983) give a good discussion of crystal growth under these conditions, they fail to point out, as Duttlinger and Knöfel (1993) do, that the equation is applicable only for low supersaturation ratios, which would not result in sufficiently high pressures to deteriorate the stone. Correns's data showed significant deviation from the theoretical values for supersaturation ratios above 1.2. The frequently cited crystallization pressures (Winkler 1975) were calculated from high supersaturation ratios (2–50) that are highly unlikely in the pore systems of a stone. A comprehensive review and discussion of this topic has been presented by Duttlinger and Knöfel (1993). Another model that attempted to include this equation (Weyl 1959) is also discussed by C. Rodriguez-Navarro and E. Doehne (1999).
A second equation for calculation of crystallization pressure was developed from a thermodynamic model by H. Wellman and A. Wilson (1965, 1968). The model predicts that crystallization will originate in larger pores, as observed by Zehnder and Arnold (1989), and that porous materials with large capillaries connected to smaller ones are the most susceptible to damage. The pressure that builds up between two such connected pores as crystallization takes place is given by the modified Laplace equation:
Equation 2.
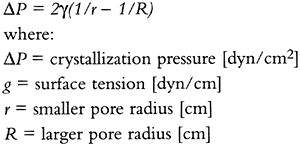 |
As Rodriguez-Navarro and Doehne (1999) discuss, the thermodynamic theory is valid only for fluid—i.e., gas-liquid or liquid-liquid systems.
B. Fitzner and R. Snethlage (1982) applied this equation to calculate crystallization pressures for four German sandstones whose pore-size distribution had been measured. Results showed that pressure should increase uniformly with diminishing pore size, in contrast to differences in actual behavior of the stones. Using pore-geometry models, they developed two different multiplying factors, V1 and V2, to correct the calculated pressures. The one that gave the best results in comparison to results of actual crystallization tests proved to be:
Equation 3.
 |
As the authors point out, this factor does not have any thermodynamic significance, but it increases the importance of smaller pores. This approach could be useful in estimating the durability of a given stone if pore-size distribution is known.
A similar approach was used by R. Rossi-Manaresi and A. Tucci (1990, 1991) to calculate the resistance to salt deterioration of different stones: three biocalcarenites, tuff, marble, and sandstone. It is interesting to note that these authors use the first correction factor, V1 = Vr/VR, which, in the case of the German sandstones, had not coincided with the experimental results. The calculations use changes of pore-size distribution in different samples of the same material—e.g., surface vs. interior. For example, the surface crust of the sandstone has a higher number of smaller pores, and hence the higher crystallization pressures should lead to its flaking. The heterogeneity in pore-size distribution of the calcarenites is postulated to produce an alveolar deterioration pattern. Finally, marble, which has few small pores, should be relatively resistant to salt crystallization. Nevertheless, Rossi-Manaresi and Tucci (1990, 1991) did not compare calculated values to actual experimental results.
7.2 6.2 HYDRATION PRESSURE
The pressure developed by volume increase upon hydration of a salt was postulated by Mortensen (1933), who proposed an equation similar to the previous one to calculate the hydration pressure:
Equation 4.
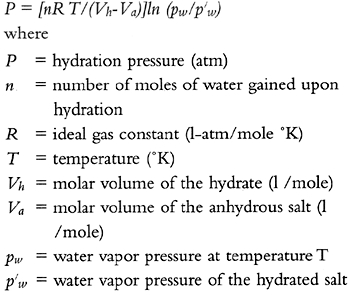 |
This approach appears logical and was not questioned for more than 60 years. Hydration pressures calculated by E. Winkler and E. Wilhelm (1970) have also been quoted extensively but, as Duttlinger and Knöfel (1993) point out, few experimental attempts to measure actual hydration pressures under controlled conditions have been undertaken. In their thorough analysis of Mortensen's hypothesis, the above equation was derived considering hydration as an osmotic process. When trying to derive it from the sources mentioned by Mortensen, Duttlinger and Knöfel (1993) obtained a slightly different equation that results in far smaller calculated pressures. Since osmotic processes require the presence of liquid water, Duttlinger and Knöfel concluded that the formation rate of the hydrate, via dissolution of the anhydrous salt and reprecipitation of the hydrate (Snethlage and Wendler 1997), must be a key factor. They suggest that the hydration rate of each individual salt could explain observed differences in “effectiveness” of the various salts.
Of the salts that are capable of hydrating, none has received so much attention as sodium sulfate because of its highly deteriorating action on stone and its use in tests to evaluate durability of stone (Price 1978; McMahon et al. 1992). Many laboratory experiments have been carried out to try to determine whether its effectiveness as a deterioration agent could be attributed either to hydration or to crystallization pressures (Chatterji et al. 1979; Sperling and Cooke 1980, 1985). Further studies on the hydration-dehydration mechanism of this salt (Charola and Weber 1992; Doehne 1994) have shown that while the dehydration of the decahydrate, mirabilite, proceeds via multiple dissolution steps ending in a polycrystalline mass of submicron particles, the hydration rate of the anhydrous salt, thenardite, is slowed down due to the formation of a hydrated film on the crystal surface. In brief, hydration of this salt does not proceed via absorption of moisture by a solid crystal but only by dissolution of that crystal and reprecipitation from solution.
7.3 6.3 OTHER MECHANISMS
J. Pühringer (1983b, 1986; Pühringer et al. 1985) suggests that other mechanisms can also contribute to the deterioration of porous materials by salts. Among them he suggests that when salt films form on the walls of pores, shear stresses can result due to changes of temperature and humidity. As Duttlinger and Knöfel (1993) point out, this situation is unlikely, because in general crystals are not directly attached to the pore wall or support. Rather they are separated by a thin solution film. However, Correns and Steinborn (1939) described how some alum crystal faces {100} grew strongly attached to glass, while other crystal faces {111} did not adhere to it. This finding may be attributed to differences in phase-boundary energies that determine whether a solution film will remain between the two materials. It is possible that some crystals could adhere to a pore wall and exert stresses on it, mainly by temperature variations, but these can at most be a minor contributing factor to the overall salt deterioration process.
S. Lewin (1990) proposes that the effectiveness of salts as deteriorating agents is related to their ability to supersaturate. He considers that when a pore space is filled by a crystalline agglomerate, the crystals themselves are most likely imperfect. Therefore it is possible for ions to diffuse into the deposit and add themselves to interior sites. This process, however, requires mechanical work, and the energy for this can be provided only if deposition occurs from a supersaturated solution. No energy can be provided by crystallization from a saturated solution since the free energy of the salt in both states—solution and solid—is the same. Lewin exemplifies this theory using marble as the porous material and a supersaturated sodium chloride (NaCl) solution (7M). Although he stresses the importance of the tendency to supersaturation that different salts have with regard to their potential as effective deteriorating agents, the ranking he presents of the supersaturation tendency of the most common salts does not conform to observed deteriorating capacities.
Finally, Snethlage and Wendler (1997) have proposed a model of dilatation and contraction under the influence of moisture and dissolved salts to explain the deterioration of clay-containing sandstones. The model considers that any deterioration process, be it physical, chemical, or biological, changes boundary surfaces that will affect intergranular contacts. Thus, the original micromorphology will be altered (Snethlage et al. 1996). The relative displacement of stone grains due to changes in moisture and the effect that dissolved salts exert on this displacement enlarge smaller pores, providing more space for salts to crystallize in, as discussed in the following section. Snethlage and Wendler (1997, 15) suggest that “The idea of a crystallization and hydration pressure should therefore still be questioned, and further research should be carried out to verify the existence of these processes as damage factors.”
7.4 6.4 FURTHER LABORATORY EXPERIMENTS
Zehnder and Arnold (1989) developed a laboratory experiment designed to grow the different crystal forms observed in situ. For this purpose they used solutions with low supersaturation ratios of various salts, including sodium chloride, sodium nitrate, sodium sulfate, and magnesium sulfate, to grow crystals on both porous and nonporous substrates. They report that the most complete crystallization sequence for a given salt was obtained at a relative humidity just below the equilibrium RH, and they differentiate four crystallization stages. These stages were essentially similar for all tested salts. Initially, crystals of nearly the equilibrium form grew within the solution and preferentially in large pores. In a second stage, as the crystals and aggregates kept growing, still covered by solution, sufficient pressures were generated to disrupt the fabric upon which they grew. This development usually occurred where pores were close together or along discontinuities in the material. In the third stage, fissures induced previously accelerated the evaporation of the solution, and, as the material dried out, fibrous crystals started to grow. During the last stage, the fibrous growth turned into thinner whisker growth that could lift flakes previously detached from the surface. Arnold and Zehnder (1990) bring together the above experiments and their previous practical experience in a second excellent summarizing paper.
Rodriguez-Navarro and Doehne (1999) developed similar crystal growth experiments but using saturated solutions of sodium chloride and sodium sulfate to try to elucidate the reason for the differences in deterioration effectiveness of these two salts. They carried out micro- to macro-experiments, and one of the most interesting results is the crystallization of these salts in capillary tubes. While sodium chloride (halite) crystallized at the air-solution interface as well as within the solution, sodium sulfate decahydrate (mirabilite) crystallized only within the bulk of the solution. In the micro- and macro-experiments it was found that the growth rate of halite crystals was much slower than that of mirabilite. The different crystal shapes obtained for halite—ranging from perfect cubic crystals when grown within the solution, to hopper-shaped or dendritic growth when formed at the air-solution interface—agree with results obtained by Zehnder and Arnold (1989). Both mirabilite and thenardite, anhydrous sodium sulfate, grew as prismatic crystals within the solution at high (65%) and low (35%) RH, respectively. The distribution of the salts within the pores of the stones also differed. Halite filled smaller pores, while both mirabilite and thenardite filled the larger pores.
Another interesting experiment developed by C. Grossi et al. (1997) takes into account the polymorphism of the anhydrous sodium sulfate, of which five phases are known. Phase V is the familiar thenardite. In the experiment, acoustic emissions were measured, particularly in larger-pored stones, that indicated stresses in the stone were induced by phase transitions since the stone had dried out completely (@ 60°C) during the test. Smaller-pored stones retained some moisture and mirabilite crystallized during the cooling stage, but no acoustic emission was obtained. Although the test temperature is above that normally found in historic buildings, the phase-transition stresses for thenardite might have relevance for rock weathering in desertic areas.
8 7. DETERIORATION PATTERNS
Conditions required for surface and subsurface crystallization of sodium chloride, leading to flaking or powdering of porous material, have been clearly described by Lewin (1982). These rely on an equilibrium between the rates of capillary migration and evaporation, and Lewin postulated that the necessary condition for this type of decay is the development of a steady state at the exposed surface. Since capillary migration is not the only mechanism involved in bringing water and salts into a porous material, a steady state is hard to imagine given the variability in environmental conditions that may lead to condensation and moisture-retention phenomena. These may play a substantial role in the amount of moisture present in the material which in turn may enhance the aging of the salt deposits influencing the deterioration pattern (Arnold and Zehnder 1985, Delgado Rodrigues 1991).
The two most common weathering patterns that can be found on any type of porous material are flaking (or contour scaling) and powdering (or sanding). Although the patterns in different materials may be similar and salts have always been reported, mechanisms that produce deterioration may vary from material to material and among salts of different solubililities. For example, in the case of clay-bearing sandstones, such as the ubiquitous brownstone in New York City, it has been shown that the deterioration may be attributed to the effect that soluble salts have on the normal hygric dilatation of these sandstones. While both hygric and hydric dilatation have been proved reversible in short-term cycling (Snethlage and Wendler 1997), the presence of soluble salts changes this behavior significantly and irreversibly (Wendler and Rückert-Thümling 1992). When salts such as sodium chloride, magnesium sulfate, and calcium nitrate are present, a stone will contract rather than expand in a moist atmosphere during cycling between 35% and 90% RH. This behavior has been attributed to the effect ions have in modifying the thickness of the double layer formed on the surface of clay minerals. Furthermore, salts concentrate in the area that retains moisture longer—i.e., the zone of maximum moisture content. From numerical model calculations, this area has been shown to be located 1–5 cm from the surface, depending on the porosity of the stone (Wendler 1991, Snethlage and Wendler 1997). This zone is mechanically stressed, leading to disrupture and eventual powdering with the detachment of surface flakes. The presence of less-soluble salts, such as gypsum, exerts a mechanical wedge action through precipitation in interstitial pores, contributing to the irreversibility of the dilatation process (Wendler and Rückert-Thümling 1992).
The mechanism resulting in flaking and sanding of porous calcareous stones appears to be different, particularly for stones exposed to air pollution. Acid attack, including carbonic acid in unpolluted rain, results in the migration of Ca++ ions to the surface, inducing a relative hardening by formation of a gypsum or a calcium carbonate crust. The underlying Ca++-depleted area loses mechanical strength and powders, following a mechanism similar to that described for tooth decay (Cussler and Featherstone 1981). This result was clearly described by W. Domaslowski (1982) and repeatedly observed in the field for more porous stones (Charola and Koestler 1985–86; Gisbert et al. 1996). However, whether the above mechanism is operative or whether a thin adherent dark gypsum layer forms on the surface is strongly dependent on the texture of the limestone, as indicated by R. Kozlowski et al. (1990). The presence of soluble salts, such as halite, can induce both flaking and sanding, depending on environmental conditions (Amadori et al. 1990). The laboratory studies of M. Amadori et al. showed that the overall porosity increased and that this salt tended to concentrate in smaller pores in the interior of the stone, as confirmed by the experiments of Rodriguez-Navarro and Doehne (1999). The presence of clays in limestones, particularly if concentrated along bedding planes, will induce delamination and scaling. This phenomenon is enhanced by the presence of salts that will contribute to increased moisture absorption (Rodriguez-Navarro et al. 1997).
The formation of gypsum crusts on low-porosity calcareous stones, such as marbles and compact limestones, has been addressed by Camuffo et al. (1982, 1983, 1987). A gypsum crust is formed by attack from acidic pollutants, and it is suggested that differences in thermal expansion between gypsum and the calcite crystals favor disruption of stone surfaces. The crystallization of gypsum between grains and in fissures provides a mechanical wedge action that leads to flaking. Soluble salts, such as halite, can also induce some flaking, but their presence results mainly in sanding (Amadori et al. 1990). Sugaring, as it is more commonly called in the case of marbles, is strongly influenced by the anisotropic behavior of the calcite under thermal stresses (Zezza et al. 1985). The presence of clays in compact limestones may give rise to different weathering patterns depending on their distribution within the limestone matrix. If present in layers, flaking and scaling will result, but if present in discrete pockets, an alveolar pattern may develop (Aires-Barros et al. 1998).
In the case of granites, flaking has been correlated to the crystallization of gypsum originating in mortars, particularly in areas where the stone remains moist for a longer time (Rivas Brea et al. 1994). On the other hand, sanding has been attributed to the presence of soluble salts as well as stresses induced during tooling of surfaces (Silva et al. 1994). Further studies have shown that flaking can occur in both fairly sound and weathered granites, while sanding occurs only in the latter. While deterioration is considered a physical process, influenced by temperature and hydric behavior among other factors, increased weathering corresponds to increased chemical changes such as leaching of key elements and oxidation-reduction reactions (Delgado Rodrigues 1996).
9 8. DISCUSSION
From the above examples it is clear that salts may not always be the original cause of deterioration, but it is certain that their presence increases the deterioration rate significantly. The key factor for the effectiveness of salts as deteriorating agents is the presence of water and/or moisture. While moisture may also substantially diminish the mechanical resistance of some stones, its distribution within the porous material determines where deterioration occurs. This area corresponds to the zone of maximum moisture content (Snethlage and Wendler 1997) and refers not only to the damage at the base of a wall with rising damp, as described by Arnold (1982) but also to the formation of gypsum veils at the top of the rising damp area, as discussed by Zehnder (1993, 1996).
As Rodriguez-Navarro and Doehne (1999) point out, crystallization will depend on both the physical properties of the salt solution, such as water vapor pressure, and its interfacial tension with the pore system of the stone. Although changes of crystal habit through the presence of substances that affect the contact angle between substrate and water have long been observed and studied, the implication this phenomenon may have in the conservation of porous materials has been discussed in relatively few papers. Pühringer has repeatedly mentioned this effect and its possible application for salt extraction or prevention of salt growth (Pühringer and Engström 1985; Pühringer 1986). The changes in morphology of gypsum crystals as a result of the application of consolidating and/or water repellent treatments was pointed out by Tucci et al. (1985), while G. Grassegger and S. Adam (1994) discussed the spiral growth of sodium nitrate in the presence of silicate ester gels, presumably due to the presence of heavy metals, such as the tin-bearing catalyst of the consolidating product. These observations were made in laboratory studies, but field observations, such as the detailed study by J. Gisbert et al. (1996), have not reported any influence of the presence of extraneous materials—for example, fly ash, micro-organisms, or biofilms—on the shape and size of the gypsum crystals present in the surface crust of a calcareous stone.
10 9. CONCLUSIONS
The present overview aimed to give a brief state-of-the-art report from the wealth of publications that have addressed salts in the deterioration of porous materials. It has clearly shown that the deterioration of porous materials induced by salt crystallization cannot be explained by a single mechanism. It has also made clear that the presence of water and/or moisture in the porous material is as important as the type of salt and the nature, texture, porosity, and interior surface of the material. Moisture transport within a pore system and the changes resulting from variations in environmental conditions—i.e., temperature and RH—are key to understanding the development of the observed deterioration patterns. The synergism that occurs among porous material, salt, and moisture will lead to the “moisture-salt-spiral” (Snethlage et al. 1996, 129) of increasing deterioration rates.
ACKNOWLEDGEMENTS
This article was developed through a US/ICOMOS contract under a grant from the U.S. National Park Service and the National Center for Preservation Technology and Training. Its contents are solely the responsibility of the author and do not necessarily represent the official position or policies of the National Park Service or the National Center for Preservation Technology and Training.
REFERENCES
Aires-Barros, L., M. J.Basto, R. C.Graça, A.Dionisio, J.Delgado Rodrigues, F. M. A.Henriques, and A. E.Charola. 1998. Stone deterioration on the tower of Belem. International Journal for Restoration of Buildings and Monuments4(6):611–26.
Amadori, M.L. L.Lazzarini, and S.Massa. 1990. Il deterioratmento da sodio cloruro di rocce compatte e porose a Venezia. In First International Symposium on the Conservation of Monuments in the Mediterranean Basin, ed. F.Zezza. Brescia: Grafo. 83–89.
American college dictionary. 1962. New York: Random House. S.v. “hygroscopicity.”
Amoroso, G. G., and V.Fassina. 1983. Stone decay and conservation. Amsterdam and New York: Elsevier.
Arnold, A. 1982. Rising damp and saline minerals. In Fourth International Congress on the Deterioration and Preservation of Stone Objects, ed. K. L.Gauri and J. A.Gwinn. Louisville, Ky.: University of Louisville. 11–28.
Arnold, A., and A.Kueng. 1985. Crystallization and habits of salt efflorescences on walls, I, Methods of investigation and habits. In Fifth International Congress on the Deterioration and Conservation of Stone, ed. G.Félix. Lausanne: Presses Polytechniques Romandes. 255–67.
Arnold, A., and K.Zehnder. 1985. Crystallization and habits of salt efflorescences on walls II. Conditions of crystallization. In Fifth International Congress on the Deterioration and Conservation of Stone, ed. G.Félix. Lausanne: Presses Polytechniques Romandes. 269–77.
Arnold, A., and K.Zehnder. 1988. Decay of stony materials by salts on humid atmosphere. In Sixth International Congress on Deterioration and Conservation of Stone, ed. J.Ciabach. Torun: Nicholas Copernicus University. 138–48.
Arnold, A., and K.Zehnder. 1990. Salt weathering on monuments. In First International Symposium on the Conservation of Monuments in the Mediterranean Basin, ed. F.Zezza. Brescia: Grafo. 31–58.
Behlen, A., M.Steiger, and W.Dannecker. 1997. Quantification of the salt input by wet and dry deposition on a vertical masonry. In Fourth International Symposium on the Conservation of Monuments in the Mediterranean Basin, ed. A.Moropoulou et al. Rhodes and Athens: Technical Chamber of Greece. 237–46.
Camuffo, D.1998. Microclimate for cultural heritage. Amsterdam and New York: Elsevier.
Camuffo, D., M.Del Monte, C.Sabbioni, and C.Vittori. 1982. Wetting, deterioration and visual features of stone surfaces in an urban area. Atmospheric Environment16(9):2253–59.
Camuffo, D., M.Del Monte, and C.Sabbioni. 1983. Origin and growth mechanisms of the sulfated crusts on urban limestone. Water, Air and Soil Pollution19:351–59.
Camuffo, D., M.Del Monte, and C.Sabbioni. 1987. Influenza delle precipitazioni e della condensazione sul degrado superficiale dei monumenti in marmo e calcare. Materiali Lapideisupp. to the Bolletino d'Arte, no. 41. Rome: Ministero per i Beni Culturali e Ambientali. 15–36.
Charola, A. E., and R. J.Koestler. 1985–86. SEM study of the deterioration of monumental stones in Vienna. In Wiener Berichte über Naturwissenschaft in der Kunst, ed. A.Vendl et al.Vienna: Orac. 176–82.
Charola, A. E., and J.Weber. 1992. The hydration-dehydration mechanism of sodium sulphate. In Seventh International Congress on the Deterioration and Conservation of Stone, ed. J.Delgado Rodrigues et al.Lisbon: Laboratorio Nacional de Engenharia Civil. 581–590.
Chatterji, S., P.Christensen, and G.Overgaard. 1979. Mechanisms of breakdown of natural stones caused by sodium salts. In Third International Congress on the Deterioration and Conservation of Stone, ed. B.Badan. Padova: Università degli Studi di Padova. 131–34.
Correns, C. W.1926. Über die Erklärung der sogennanten Kristallisationskraft. Berichte der Preussischen Akademie der Wissenschaft11:81–88.
Correns, C. W.1949. Growth and dissolution of crystals under linear pressure. Discussions of the Faraday Society5:267–71.
Correns, C. W., and W.Steinborn. 1939. Experimente zur Messung und Erklärung der sogennanten Kristallisationskraft. Zeitschrift für Kristallographie101:117–33.
Cussler, E. L., and J. D. B.Featherstone. 1981. Demineralization of porous solids. Science213:1013.
Delgado Rodrigues, J.1991. Causes, mechanisms and measurement of damage in stone monuments. In Science, technology and European cultural heritage, ed. N. S.Baer et al.Oxford: Butterworth-Heinemann. 124–37.
Delgado Rodrigues, J.1996. Conservation of granitic rocks with application to the megalithic monuments. Conclusions report. In Degradation and conservation of granitic rocks in monuments. Protection and Conservation of European Cultural Heritage Research Report 5. Brussels: European Commission. 161–242.
Doehne, E.1994. In situ dynamics of sodium sulfate hydration and dehydration in stone pores: Observations at high magnification using the environmental scanning electron microscope. In Third International Symposium on the Conservation of Monuments in the Mediterranean Basin, ed. V.Fassina et al.Venice: Soprintendenza ai Beni Artistici e Storici di Venezia. 143–50.
Domaslowski, W.1982. La conservation préventive de la Pierre. Paris: UNESCO.
Duttlinger, W., and D.Knöfel. 1993. Salzkristallisation und Salzschadensmechanismen. In Jahresberichte Steinzerfall: Steinkonservierung 1991, ed. R.Snethlage. Berlin: Ernst & Sohn. 197–213.
Evans, I. S.1969–70. Salt crystallization and rock weathering: A review. Revue de Géomorphologie Dynamique19(4):153–77.
Feilden, B. M.1982. Conservation of historic buildings. London: Butterworth Scientific. 99–107.
Fitzner, B., and R.Snethlage. 1982. Einfluß der Porenradienverteilung auf das Verwitterungsverhalten asugewälter Sandsteine. Bautenschutz ± Bausanierung5(3):97–103.
Franke, L., and F.Pinsler. 1998. Untersuchungen von Saltztransportprozessen und deren Visualisierung mit Hilfe der Röntgen-analytik. International Journal for Restoration of Buildings and Monuments4(3):187–207.
Gisbert, J., C.Martin-Chaves, and N.Andaluz. 1996. Black crusts from Rueda Cloister: An approximation to fossil effects of pollution in a semi-arid climate. In Eighth International Congress on the Deterioration and Conservation of Stone, ed. J.Riederer. Berlin: Möller Druck. 387–92.
Goudie, A., and H.Viles. 1997. Salt weathering hazards. Chichester: J. Wiley & Sons.
Grassegger, G., and S.Adam. 1994. Untersuchungen zur Entwicklung der mikroskopischen Gefüge von Kieselsäureester-Gelen in Porenräumen mit und ohne Salz-, Feuchtestöreffekte. In Jahresberichte Steinzerfall: Steinkonservierung 1992, ed. R.Snethlage. Berlin: Ernst & Sohn. 127–33.
Grossi, C. M., R. M.Esbert, L. M.Suárez del Río, M.Montoto, and M.Laurenzi-Tabasso. 1997. Acoustic emission monitoring to study sodium sulphate crystallization in monumental porous carbonate rocks. Studies in Conservation42:115–25.
Henriques, F.M.A.1994. Humidade em paredes. Lisbon: Labóratorio Nacional de Engenharia Civil. 1–17.
Iñiguez Herrero, J.1967. Altération des calcaires et des grès utilisés dans la construction, trans. J. Stichelbaudt. Paris: Eyrolles. 28–45.
Kozlowski, R., J.Magiera, J.Weber, and J.Haber. 1990. Decay and conservation of Pinczow porous limestone. Part 1. Lithology and weathering. Studies in Conservation35:205–21.
Krauskopf, K. B.1979. Introduction to geochemistry. New York: McGraw-Hill.
Lewin, S. Z.1982. The mechanism of masonry decay through crystallization. In Conservation of historic stone buildings and monuments. Washington, D.C.: National Academy Press. 120–44.
Lewin, S. Z.1990. The susceptibility of calcareous stones to salt decay. In First International Symposium on the Conservation of Monuments in the Mediterranean Basin, ed. F.Zezza. Brescia: Grafo. 59–53.
Massari, G., and I.Massari. 1993. Damp buildings, old and new. Rome: ICCROM. 7–12.
McMahon, D. J., P.Sandberg, K.Folliard, and P. K.Mehta. 1992. Deterioration mechanisms of sodium sulfate. In Seventh International Congress on the Deterioration and Conservation of Stone, ed. J.Delgado Rodrigues et al.Lisbon: Laboratorio Nacional de Engenharia Civil. 705–14.
Mortensen, H.1933. Die “Salzsprengung” und ihre Bedeutung für die Regionalklimatische Gliederung der Wüsten. Dr. A. Petermanns Mitteilungen. Gotha: Justus Perthes. 79:130–35.
Price, C. A.1978. The use of the sodium sulphate crystallization test for determining the weathering resistance of untreated stone. In Deterioration and protection of stone monuments. International Symposium. Paris: UNESCO-RILEM. 3.6.
Price, C. A.1996. Stone conservation: An overview of current research. Santa Monica, Calif.: Getty Conservation Institute, J. Paul Getty Trust. 7–9.
Price, C. A., and P.Brimblecombe. 1994. Preventing salt damage in porous materials. In Preventive conservation, practice, theory and research, ed. A.Roy and P.Smith. London: International Institute for Conservation of Historic and Artistic Works. 90–93.
Pühringer, J.1983a. Salt disintegration: Salt migration and degradation by salt—A hypothesis. D15:1983. Stockholm: Swedish Council for Building Research.
Pühringer, J.1983b. Salzwanderung und Verwitterung durch Salze. In Materials science and restoration, ed. F. H.Wittmann. Filderstadt: Edition Lack + Chemie. 361–66.
Pühringer, J.1986. Mögligkeit zur Verhinderung von Schäden durch Salze. In Materials science and restoration, ed. F. H.Wittmann. Ostfildern: Technische Akademie Esslingen. 359–64.
Pühringer, J., L.Berntsson, and B.Hedberg. 1985. Hydrate salts and degradation of materials. In Fifth International Congress on the Deterioration and Conservation of Stone, ed. G.Félix. Lausanne: Presses Polytechniques Romandes. 231–40.
Pühringer, J., and L.Engström. 1985. Unconventional methods for the prevention of salt damage. In Fifth International Congress on the Deterioration and Conservation of Stone, ed. G.Félix. Lausanne: Presses Polytechniques Romandes. 241–50.
Rivas Brea, T., B.Prieto Lamas, and B.Silva Hermo. 1994. Plaque-shedding by granite in the monastery of San Martín Pinario (Santiago de Compostela, NW Spain). In Third International Symposium on the Conservation of Monuments in the Mediterranean Basin, ed. V.Fassina et al.Venice: Soprintendenza ai Beni Artistici e Storici di Venezia. 737–41.
Rodriguez-Navarro, C., and E.Doehne. 1999. Salt weathering: Influence of evaporation rate, supersaturation and crystallization pattern. Earth Surface Processes and Landforms24:191–209.
Rodriguez-Navarro, C., E.Hansen, E.Sebastian, and W. S.Ginell. 1997. The role of clays in the decay of ancient Egyptian limestone sculptures. Journal of the American Institute for Conservation36:151–63.
Rossi-Manaresi, R., and A.Tucci. 1990. Pores structure and salt crystallization: “Salt decay” of Agrigento Biocalcarenite and “case hardening” in sandstone. In First International Symposium on the Conservation of Monuments in the Mediterranean Basin, ed. F.Zezza. Brescia: Grafo. 97–100.
Rossi-Manaresi, R., and A.Tucci. 1991. Pore structure and the disruptive or cementing effect of salt crystallization in various types of stone. Studies in Conservation36:53–58.
Silva, B., M.Casal, B.Prieto, T.Rivas, and F.Guitián. 1994. Form and factors of weathering in the cathedral of Santiago de Compostela (NW Spain). In Third International Symposium on the Conservation of Monuments in the Mediterranean Basin, ed. V.Fassina et al.Venice: Soprintendenza ai Beni Artistici e Storici di Venizia. 743–48.
Snethlage, R., and E.Wendler. 1997. Moisture cycles and sandstone degradation. In Saving our architectural heritage: The conservation of historic stone structures, ed. N. S.Baer and R.Snethlage. Chichester: Elsevier. 7–24.
Snethlage, R., E.Wendler, and D. D.Klemm. 1996. Tenside im Gesteinsschutz—Bisherige resultate mit einem neuen Konzept zur Erhaltung von Denkmälern aus Naturstein. In Natursteinkonservierung in der Denkmalpflege, ed. R.Snethlage. Berlin: Ernst & Sohn. 127–46.
Sperling, C. H. B., and U.Cooke. 1980. Salt weathering in arid environments. Part 1, Theoretical considerations. Papers in Geography, no. 8. London: Department of Geography, Bedford College.
Sperling, C. H. B., and U.Cooke. 1985. Laboratory simulation of rock weathering by salt crystallization and hydration processes in hot, arid environments. Earth Surface Processes and Landforms10:541–55.
Steiger, M.1996. Distribution of salt mixtures in a sandstone monument: Sources, transport and crystallization properties. In EC Research Workshop on Origin, Mechanisms and Effects of Salts on Degradation of Monuments in Marine and Continental Environments, ed. F.Zezza. Protection and Conservation of the European Cultural Heritage. Research Report 4. Bari: European Commission. 241–46.
Steiger, M., A.Behlen, H.-H.Neumann, U.Willers, and C.Wittenburg. 1997. Sea salt in historic buildings: Deposition, transport and accumulation. In Fourth International Symposium on the Conservation of Monuments in the Mediterranean Basin. Ed. A.Moropoulou et al.Rhodes/Athens: Technical Chamber of Greece.
Steiger, M., and W.Dannecker. 1995. Hygroskopische Eigenschaften und Kristallisationsverhalten von Salzgemischen. In Jahresberichte Steinzerfall: Steinkonservierung 1993, ed. R.Snethlage. Berlin: Ernst & Sohn. 115–28.
Steiger, M., H.-H.Neumann, T.Grodten, C.Wittenburg, and W.Dannecker. 1998. Salze in Natursteinmauerwerk: Probenahme, Messung und Interpretation. In Natursteinkonservierung 2, ed. R.Snethlage. Stuttgart: Fraunhofer IRB Verlag. 61–91.
Steiger, M., H.-H.Neumann, A.Ulrich, and W.Dannecker. 1993. Chemische Zusammensetzung und Verteilung löslicher Salze in Natursteinmauerwerk. In Jahresberichte Steinzerfall: Steinkonservierung 1991, ed. R.Snethlage. Berlin: Ernst & Sohn. 21–33.
Steiger, M., and A.Zeunert. 1996. Crystallization properties of salt mixtures: Comparison of experimental results and model calculations. In Eight International Congress on the Deterioration and Conservation of Stone, ed. J.Riederer. Berlin: Möller Druck. 535–44.
Tucci, A., R. J.Koestler, A. E.Charola, and R.Rossi-Manaresi. 1985. The influence of acid rain and UV radiation on the ageing of acrylic and silicone resins. In Fifth International Congress on the Deterioration and Conservation of Stone, ed. G.Félix. Lausanne: Presses Polytechniques Romandes. 891–98.
Vos, B. H.1971. Suction of groundwater. Studies in Conservation16:129–44.
Weber, H.1984. Mauerfeuchtigkeit. Sindelfingen, Germany: Expert Verlag. 15–37.
Wellman, H. W., and A. T.Wilson. 1965. Salt weathering: A neglected geological erosive agent in coastal and arid environments. Nature205(4976):1097–98.
Wellman, H. W., and A. T.Wilson. 1968. Salt weathering or fretting. In Encyclopedia of geomorphology, ed. R. W.Fairbridge. Stroudsburg, Pa.: Dowden, Hutchinson & Ross. 968–70.
Wendler, E.1991. Zum Mechanismus der Schalenbildung bei tonigen Sandsteinen. Jahresberichte Steinzerfall: Steinkonservierung 1989, ed. R.Snethlage. Berlin: Ernst & Sohn. 71–76.
Wendler, E., and R.Rückert-Thümling. 1992. Gefügezerstörendes Verformungsverhalten bei salzbefrachteten Sandsteinen unter hygrischen Wechselbelastung. In Materials science and restoration, ed. F. H.Wittmann. Ehningen bei Böblingen, Germany: Expert Verlag. 1818–30.
Weyl, P. K.1959. Pressure solution and the force of crystallization: A phenomenological theory. Journal of Geophysical Research64(11) 2001–25.
Winkler, E. M.1975. Stone: Properties, durability in man's environment. 2d ed. Vienna and New York: Springer Verlag.
Winkler, E. M., and E. J.Wilhelm. 1970. Salt burst by hydration pressures in architectural stone in urban atmosphere. Bulletin of the Geological Society of America81:567–72.
Zehnder, K.1993. New aspects of decay caused by crystallization of gypsum. In Conservation of stone and other materials.Vol. 1. Causes of disorders and diagnosis, ed. M.-J.Thiel. London: E & FN Spon. 107–14.
Zehnder, K.1996. Gypsum efflorescences in the zone of rising damp: Monitoring the slow decay processes caused by crystallizing salts on wall paintings. In Eighth International Congress on the Deterioration and Conservation of Stone, ed. J.Riederer. Berlin: Möller Druck. 1669–78.
Zehnder, K., and A.Arnold. 1989. Crystal growth in salt efflorescence. Journal of Crystal Growth97:513–21.
Zezza, U., E.Previde Massara, V.Massa, and D.Venchiarutti. 1985. Effect of temperature on intergranular decohesion of the marbles. In Fifth International Congress on the Deterioration and Conservation of Stone, ed. G.Félix. Lausanne: Presses Polytechniques Romandes. 131–40.
AUTHOR INFORMATION
A. ELENA CHAROLA obtained her degree in chemistry from the Universidad Nacional de La Plata in her native country, Argentina. She completed her postdoctoral work at New York University and stayed in the United States, becoming a citizen 20 years ago. She served as associate chemist in the Department of Objects Conservation at the Metropolitan Museum of Art, New York City, scientific adviser at ICCROM (International Centre for the Study of the Preservation and Restoration of Cultural Property) in Rome, Italy, and consultant to the Easter Island Program for World Monuments Fund (WMF). Since her return to the United States some 10 years ago, she has continued as technical consultant to WMF, including projects in other countries (Torre de Belem and Mosteiro dos Jerónimos, Lisbon, Portugal, and Belvedere, Vienna, Austria) while being an independent consultant in conservation. She has been lecturer in advanced architectural conservation in the Graduate Program in Historic Preservation, University of Pennsylvania, and national chair of the Brick Masonry Committee of US/ICOMOS since 1996. Address: 3618 Hamilton St., Philadelphia, Pa. 19104
Received for review March 3, 2000. Revised manuscript received April 29, 2000. Accepted for publication May 15, 2000.
Section Index |