INFRARED MICROSPECTROSCOPY USING A SYNCHROTRON SOURCE FOR ARTS-SCIENCE RESEARCH
GREGORY D. SMITH
ABSTRACT—The nature of radiation from synchrotron storage rings is discussed with emphasis on the advantages, in particular that of brightness, that this light source offers when replacing the thermal source typically employed in a conventional infrared microscope. Specifically, synchrotron radiation allows the collection of high signal-to-noise ratio infrared spectra with diffraction-limited spatial resolution across the entire near-, mid-, and far-infrared spectral range. This level of performance permits the spatially resolved analysis of microscopic samples from artwork and artifacts down to ~ 4 μm in the mid-infrared spectral region and potentially much smaller dimensions, for both organic and inorganic components. Conservation and archaeological scientists are alerted to the worldwide availability of these microscopy facilities for occasional use by museums and galleries free of charge.
TITRE—La microspectroscopie infrarouge � l'aide d'une source lumineuse synchrotronique dans la recherche en science des arts. R�SUM�—La nature du rayonnement synchrotron �mise par ce genre d'acc�l�rateur de particules est discut�e en mettant l'accent sur ses avantages, en particulier le degr� de luminance (intensit� lumineuse par unit� de surface) du faisceau, ce qui pourrait le rendre utile pour remplacer les sources thermiques de lumi�re typiquement employ�es dans les microscopes � l'infrarouge conventionnels. En particulier, le rayonnement synchrotron permet de recueillir un spectre infrarouge ayant un rapport signal/bruit �lev� et dont la limite de r�solution spatiale est celle d'un faisceau diffractionnel id�al sur toute la plage infrarouge (infrarouge proche, moyen et lointain). Ce niveau de rendement permet l'analyse � haute limite de r�solution spatiale d'�chantillons de dimensions microscopiques, c'est-�-dire de 4 microns dans le domaine spectral de l'infrarouge moyen et potentiellement beaucoup plus petits encore, provenant d'objets culturels ou d'oeuvres d'art, compos�s tant de mati�re organique qu'inorganique. Les scientifiques en restauration et en arch�om�trie sont appel�s � utiliser ces �quipements en microscopie, qui sont disponibles � l'�chelle internationale et sans frais pour usage occasionnel au profit de mus�es et autres institutions culturelles.
TITULO—Microespectroscop�a infraroja con fuente de sincrotr�n para la investigacion cient�fica de las artes. RESUMEN—El art�culo discute la naturaleza de la radiaci�n emitida por aros de almacenamiento de sincrotr�n con �nfasis en sus ventajas. En particular, la del brillo que ofrece esta fuente de luz cuando se la utiliza en reemplazo de la fuente t�rmica t�picamente empleada en microscop�a infrarroja convencional. Espec�ficamente, la radiaci�n del sincrotr�n permite el registro de espectros infrarrojos con alta relaci�n se�al-ruido y con resoluci�n espacial limitada por difracci�n a trav�s de todo el rango espectral infrarrojo cercano, medio y lejano. Este nivel de rendimiento permite el an�lisis de muestras microsc�picas de obras de arte y artefactos con una resoluci�n espacial de hasta ~ 4 μm en la regi�n espectral del infrarrojo medio y, potencialmente, en dimensiones mucho m�s peque�as, tanto para componentes org�nicos como inorg�nicos. Se alerta a los cient�ficos en las �reas de conservaci�n y arqueolog�a sobre la disponibilidad a nivel mundial de estos micrsocopios para uso ocasional de museos y galerias de forma gratuita.
T�TULO—Micro-espectrocopia infravermelha utilizando uma fonte sincrotr�nica em pesquisas cient�ficas de arte. RESUMO—A natureza da radia��o proveniente dos an�is de armazenamento sincrotr�nicos � analisada neste artigo, enfatizando as vantagens, especialmente da luminosidade, que este tipo de fonte de luz oferece ao substituir a fonte t�rmica tipicamente utilizada em um microsc�pio infravermelho convencional. Especificamente, as radia��es sincrotr�nicas permitem a aquisi��o de espectros no infravermelho de elevada rela��o sinalru�do com resolu��o espacial limitada por difra��o, ao longo de toda a faixa espectral do infravermelho pr�ximo, m�dio e long�nquo. Este n�vel de desempenho possibilita a an�lise espacialmente resolvida de amostras microsc�picas de obras de arte e artefatos de at� 4 μm na regi�o espectral do infravermelho m�dio e dimens�es potencialmente muito menores, tanto para componetes org�nicos quanto inorg�nicos. Cientistas da �rea da conserva��o e arque�logos s�o alertados para a disponibilidade a n�vel mundial destes recursos em microscopia para utiliza��o ocasional gratuita por parte de museus e galerias.
1 INTRODUCTION AND DISCUSSION
Fourier transform infrared (FTIR) microscopy has been widely applied to the chemical analysis of microscopic samples in conservation science and archaeometry.1 This spectroscopic technique combines light microscopy's ability to resolve the microstructural details of a sample with the molecular specificity of infrared (IR) spectroscopy for identifying its heterogeneous chemical components. A schematic of a conventional IR microscope employing Schwarzschild reflecting optics is shown in figure 1 (see page 441 for color image). An IR source, typically a centimeter-sized thermal radiator at ~ 1200 K, emits broadband radiation in all directions (4π steradians), a small portion of which (~ 1%) is captured by the instrument. This radiation is passed to an interferometer, where it is spectrally encoded, and then on to a microscope objective that focuses the light onto the sample. If the sample is sufficiently thin, the portion of light transmitted by the sample is collected by a condensing optic and directed to the detector as shown in figure 1; otherwise, the light reflected from the surface of the sample can be collected by the original focusing optic and sent via an alternate path to the detector (not shown).
In either instance, a ratio of the background detector signal and the signal with the sample in the beam determines the transmission or reflection spectrum of the material. Chemical maps of heterogeneous samples can be produced by rastering the
Fig. 1.
Schematic of a conventional IR microscope operating in transmission mode and employing Schwarzschild reflecting optics and confocal apertures. This is a modified version of original artwork appearing in Carr 2001 (see page 441 for color image).
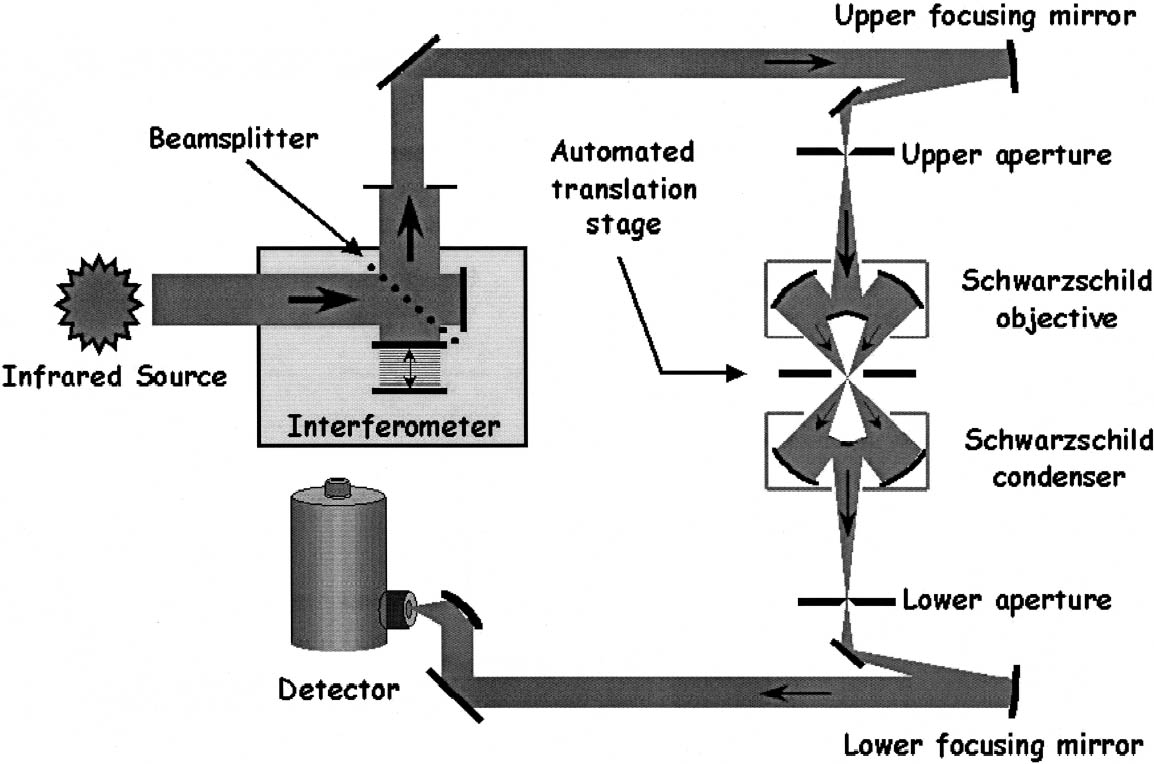 |
sample through the beam in a point-by-point fashion and collecting a full IR spectrum at each position. The power of this technique for identifying and determining the lateral dimensions of distinct chemical domains in a sample has been described by Martoglio Smith (2000).
The ability of the focusing optics to illuminate selectively a minute area is directly related to the source size in a conventional IR microscope, and a typical instrument achieves a source image at the sample of ~ 100 μm. In order to restrict the sampled area to smaller dimensions, an upper field stop aperture (see fig. 1) is used to mask the IR beam at a remote image plane prior to the microscope objective, effectively reducing the apparent size of the source. Unfortunately, limiting the �tendue, or light throughput, in this way also degrades the signal-to-noise ratio (S/N) of the spectrum, and practical limits are reached at aperture dimensions (and therefore sampled area dimensions) of ~ 20 � 20 μm. Simply put, the source brightness—i.e., the photon flux per unit area—is too small for adequate detection if the illuminated spot on the sample or the sample itself is reduced beyond this value.
This limitation precludes the spatially resolved analysis of sample dimensions of prime importance in the examination of historical materials, namely those < 20 μm. One example of a common sample with interesting chemical heterogeneity below the ~ 20 μm limit would be a typical painting cross section consisting of chemically distinct ground, paint, glaze, and varnish layers. Similarly, the paint mixtures themselves are often composed of numerous pigments, driers, and extenders whose individual grain sizes can often be far less than 20 μm. The spectrum collected from these samples by a conventional IR microscope would be a complicated convolution of all the component materials' absorption features.
Raman microscopy, a complementary vibrational spectroscopic technique, is increasingly being used to fill this resolution gap in conservation science (Smith and Clark 2001). The Raman microscope relies on inelastic laser scattering to measure a vibrational spectrum, and the excitation lasers that are used can be focused easily to ~ 1 μm. Unlike IR spectroscopy, though, Raman analysis can be frustrated by sample fluorescence (Bell et al. 2000), photothermal degradation of the sample (Burgio et al. 2001; Smith et al. 2001), and weak Raman scattering from some materials, especially organic compounds (Bruni et al. 1999). In comparison, IR spectroscopy does not suffer from fluorescence, and, in fact, it is routinely used in combination with low concentration fluorescent probes that are used to stain biological tissues (Miller and Tague 2002). Furthermore, although IR synchrotron radiation (IRSR) can be orders of magnitude brighter than conventional IR sources (see below), the former has not been shown to cause any sample damage (Martin et al. 2001), even when analyzing sensitive living cells (Jamin et al. 1998).
Synchrotron radiation from an electron storage ring eases the restrictions on spatial resolution for IR microscopy by providing a high-flux, highly collimated, broadband2 source of photons (Roy et al. 2001). These photons are emitted by “bunches” of electrons as they pass through high-field bending magnets that maintain the electrons' orbit around the ring. The fact that the radiation is generated by relativistic particles confined to a precise orbit means that the “source” size is intrinsically small. The combination of small source size, high collimation, and large photon flux translates into extremely high brightness and therefore quicker data collection, higher spatial resolution, and greater S/N for an �tendue-limited experiment such as IR microscopy. IRSR beamlines often incorporate as the experimental station a commercial IR microscope that has had the thermal source removed or bypassed in order to accommodate the IRSR beam (Carr et al. 1998). Therefore, performing an IRSR microscope measurement is similar experimentally to using a conventional instrument, and no further complications are introduced by utilizing the brighter source.
Bending magnet IRSR represents a near-ideal point source in the mid- to far-IR region, such that the spatial resolution is no longer limited by the size of the source but by diffraction effects. The diffraction-limited illumination pattern of such a source when imaged onto a sample, often referred to as the point spread function (PSF), depends on the wavelength of the light, λ, the refractive index of the medium in which the measurement is being made, n, and the sine of the half-angle acceptance of the microscope optic, θ (Hecht and Zajac 1974). The PSF of a typical Schwarzschild reflecting objective has been calculated by Carr (2001) and compared to that of a common refractive optic. The Rayleigh criterion—i.e., the spatial distance separating the first minima of the PSF—for a Schwarzschild objective can be expressed as
Table (1.1).
 |
where sin θ is often approximated as the numerical aperture (NA) of the optic. This equation has been verified experimentally at the National Synchrotron Light Source (NSLS) for a commercial IR microscope (NA = 0.6) using an IRSR source and measuring in air (n = 1); the full width at half maximum of the central cone of intensity for 6 μm radiation (1666 cm-1) has been determined to be ~ 4 μm (Carr 2001). When employed in a confocal geometry—i.e., with a second aperture of the same dimension located at a remote image plane after the sample and before the detector (see fig. 1), the IRSR microscope performs considerably better due to improved rejection of light from areas outside the masked sample area; for the same experimental conditions, the distance between first minima falls to ~ 2 μm (Carr 2001). It is important to note, however, that the effective spatial resolution for these reflecting optics is somewhat poorer due to the diffraction rings caused by the obscuration of the nested mirror in a Schwarzschild objective (see fig. 1). These diffraction rings contain significant intensity (~ 50%), and so the effective spatial resolution, defined as the distance over which the signal changes from 10% to 90% when the focused light is scanned across a sharp-edge target, is ~ 2λ when using a single aperture and 30% better in a confocal geometry.
Figure 2 compares the theoretical brightness across the IR spectral range for a 1200 K thermal source (bottom line)—i.e., the equivalent of a conventional globar—and the typical output of a bending magnet beamline (top line) at the NSLS (Carr et al. 1998). In the mid- to far-IR, the brightness advantage of IRSR over the conventional IR source is roughly 200!
Figure 3 shows the practical results of this brightness advantage. As a simple but relevant illustration, a single grain of anhydrite (CaSO4), an artist's material commonly used in painting grounds and as a paper filler, was placed on a 1 mm thick IR-transparent substrate and analyzed in transmission using a conventional thermal source and then an IRSR source. The pigment grain was approximately 3 μm in diameter. A single upper aperture was set at the same dimensions in order to mask the particle. As shown in figure 3, the spectral differences are manifest.
This result highlights the advantages of IRSR for examining minute quantities of artists'materials, in this case a particle that is much smaller than the diffraction limit of the ~ 1150 cm-1 (λ = 8.7 μm) sulfate doublet shown prominently in the IRSR spectrum. Curiously, the two overlapping bands at ~ 3600 cm-1 and the single feature at 1615 cm-1, which are indicative of coordinated water molecules, should not be present in anhydrite. A comparison of the IRSR spectrum in figure 3 to a reference spectrum of gypsum (CaSO4•2H2O) showed that the sample had in fact become hydrated (Ferraro 1982). Since the sample preparation was done quickly and the IR microscope system remained purged with dry nitrogen throughout the course of the measurements, the hydration of the sample was most likely a result of the storage container's having been left open to air at some point.
Importantly, the previous example required no sample preparation. This would be the case, for instance, when analyzing small samples of material taken from paintings or artifacts, provided they were thin or could be crushed and were not highly absorbing. For thicker samples (e.g., embedded painting cross sections) or those materials that absorb strongly (e.g., dark polymers), micro-attenuated total internal reflection (μ-ATR) objectives are able to collect excellent-quality spectra from just the surface of the sample by directly contacting it. For specularly reflective materials, a noncontacting reflection mode is widely employed, although the resulting reflectance spectrum requires mathematical manipulation in order to generate the more familiar absorption spectrum.
All of these accessories and measurement modes are commonly used in conventional IR microscopy and are similarly available at IRSR facilities. Additionally, although the spectra above were collected in the mid-IR (4000–600 cm-1), which is the spectral range commonly accessible to conventional IR microscopes, the facilities at the NSLS allow diffraction-limited IR microscopy in the far-IR (600–50 cm-1) as well, where many metal oxides and sulfides have characteristic molecular vibrations and crystal phonon modes. This increased spectral range adds to the number of inorganic materials that can be analyzed using IRSR microscopy, although at these longer wavelengths the diffraction-limited spot size increases significantly, thus degrading the spatial resolution of the analysis.
Fig. 2.
Calculated brightness across the IR spectral region for a 1200 K blackbody thermal source (bottom line) and for synchrotron bending-magnet radiation from the NSLS (top line) extracted from a solid angle of 90 � 90 mrad2 at 800 mA electron beam current
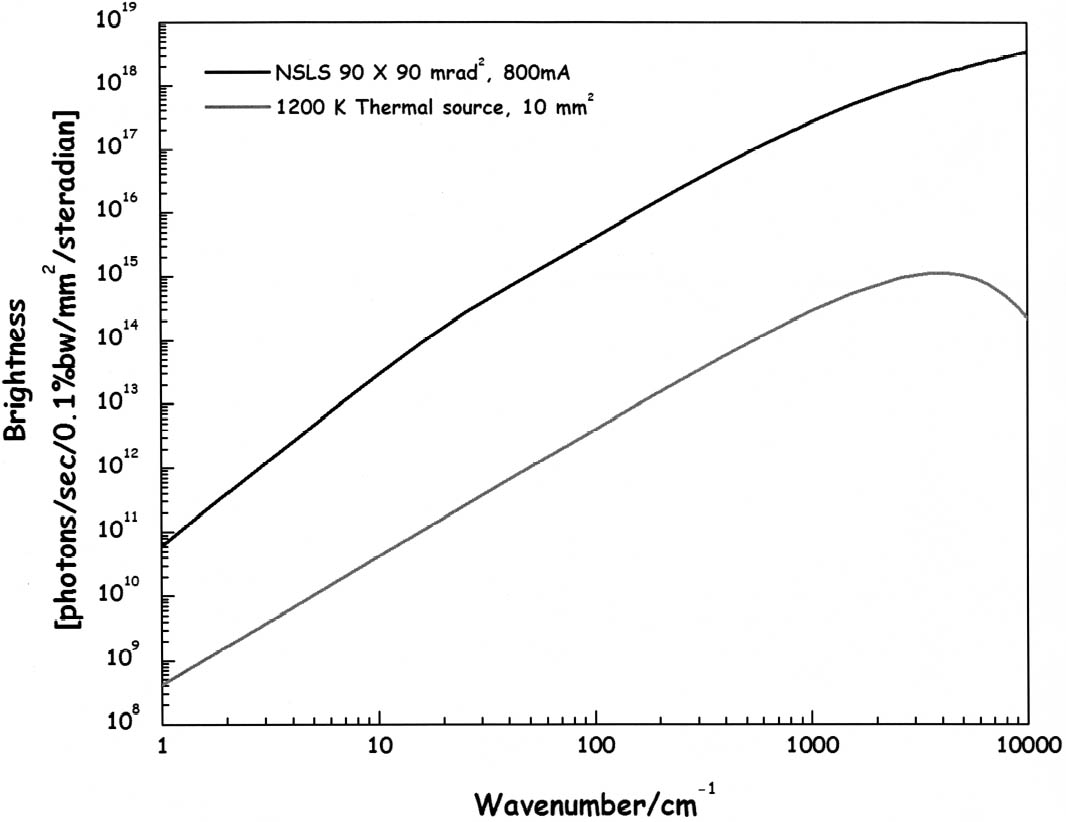 |
Fig. 3.
Baseline-corrected IR transmission spectra of a 3 μm diameter anhydrite (CaSO4) particle on a 1 mm thick IR-transparent substrate. The spectra were measured with a conventional thermal source (gray line) and with IRSR (black line) from the U4IR beamline at the NSLS. The sample was masked by a single 3 � 3 μm upper aperture, and the transmitted light was detected with an MCT-A detector. The spectra are an average of 256 scans at 8 cm-1spectral resolution. A comparison of the IRSR spectrum with a reference spectral library showed that the anhydrite sample had in fact become hydrated to form gypsum (CaSO4•2H2O).
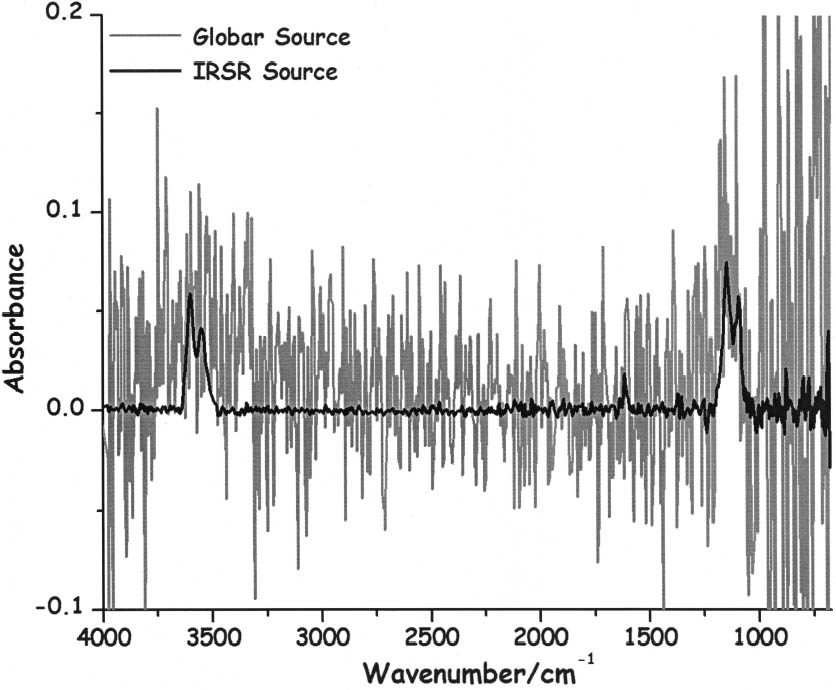 |
Current research at the NSLS seeks to improve further the spatial resolution of IRSR microscopy through the use of contact optics made of high n materials, such as zinc selenide, silicon, diamond, and germanium. This approach is identical to that of oil immersion optics used in visible light microscopy (Hecht and Zajac 1974), except that the crystals here provide a solid, IR-transparent alternative to oils. By performing measurements through these materials, improvements in spatial resolution (see equation 1.1) of between 2.4 and 4 across the entire IR spectral range can be realized (Sommer et al. 2001).
Synchrotron radiation facilities are operated largely by academic institutions and national governments as “user” facilities, meaning that a major portion of their operating time is reserved for use by outside scientists. To be eligible, interested parties submit proposals for research projects and are awarded the appropriate block of “beamtime” in which to run their experiments. Table 1 lists many of the synchrotron laboratories with operational or planned beamlines dedicated to IRSR microscopy. The use of these facilities, their instrumentation, and the scientific expertise of the beamline operators is free of charge to qualified users pursuing nonproprietary research. In some instances, funds are even available for travel and short stays at the site. These conditions and the location of numerous facilities within a short distance of major metropolitan museums around the world make synchrotron laboratories an excellent resource for conservation and archaeological scientists. At these facilities, arts-based science researchers can gain access to state-of-the-art instrumentation for performing collaborative research projects or the occasional analysis of particularly complicated specimens where the high spatial resolution and extended spectral range of IRSR microscopy are required.
2 CONCLUSIONS
Numerous research groups have already benefited from the advantages of synchrotron radiation when used with conventional spectroscopic techniques such as x-ray diffraction (XRD) and x-ray fluorescence (XRF) for the analysis of historical materials (Salvad� et al. 2002; Veiga and Figueiredo 2002). The relatively recent interest in and development of IRSR microscopy beamlines at synchrotron facilities around the world heralds new opportunities for improvements in IR microscopy research at the arts-science interface. The low cost, proximity, and cutting-edge instrumentation of these facilities, once widely publicized, will likely prove irresistible to conservation and archaeological scientists faced with increasingly demanding scientific problems and limited budgets.
ACKNOWLEDGEMENTS
Dr. G. Lawrence Carr is recognized for providing an instrument schematic, a modified version of which forms figure 1, and for introducing the author to IRSR microscopy. Dr. Gwyn Williams is acknowledged for his original calculations of the theoretical brightness graphs shown in figure 2. The author's work was supported by the Laboratory-Directed Research and Development program at the National Synchrotron Light Source (NSLS). The NSLS is a user facility open to all qualified researchers and is funded by the U.S. Department of Energy under contract DE-AC02-98-CH10886. Information can be found at http://nslsweb.nsls.bnl.gov/infrared, and interested parties should contact Dr. G. Lawrence Carr (carr@bnl.gov) or Dr. Lisa Miller (lmiller@bnl.gov) for additional information.
NOTES
1. See, for example, the meeting proceedings of the Infrared and Raman Users Group (IRUG) at www.irug.org.
Table .
Locations of “User-Based” Synchrotron Facilities That Offer IRSR Microscopy or Are Currently Developing IRSR Microscopy Beamlines
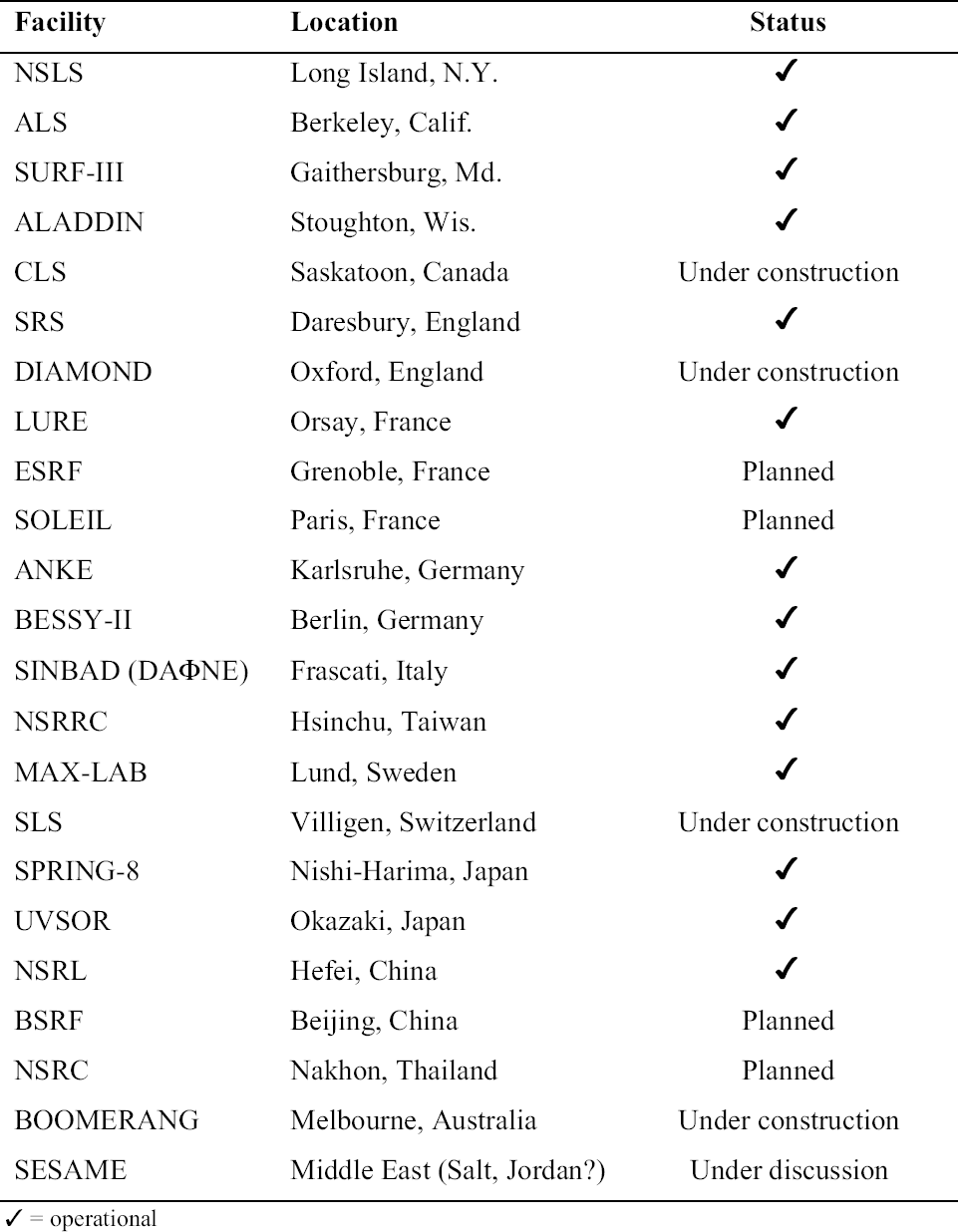 |
2. The broadband emission spans the hard x-rays to millimeter waves, although only those photons in the visible to far-IR regions are transmitted to the IRSR experimental station.
REFERENCES
Bell, S. E. J., E. S. O.Bourguignon, A. C.Dennis, J. A.Fields, J. J.McGarvey, and K. R.Seddon. 2000. Identification of dyes on ancient Chinese paper samples using the subtracted shifted Raman spectroscopy method.Analytical Chemistry72:234–39.
Bruni, S., F.Cariati, F.Casadio, and L.Toniolo. 1999. Identification of pigments on a XV century illuminated parchment by Raman and FTIR microscopies.Spectrochimica Acta A55:1371–77.
Burgio, L., R. J. H.Clark, and S.Firth. 2001. Raman spectroscopy as a means for the identification of plattnerite (PbO2), of lead pigments and of their degradation products.Analyst126:222–27.
Carr, G. L.2001. Resolution limits for infrared microspectroscopy explored with synchrotron radiation.Review of Scientific Instruments72:1–7.
Carr, G. L., P.Dumas, C. J.Hirschmugl, and G. P.Williams. 1998. Infrared synchrotron radiation programs at the National Synchrotron Light Source.Il Nuovo Cimento de Societa Italiana di Fisica D20:375–95.
Ferraro, J. R., ed.1982. The Sadtler infrared spectra handbook of minerals and clays. Philadelphia: Sadtler Research Laboratories.
Hecht, E., and A.Zajac. 1974. Optics. Reading, England: Addison-Wesley.
Jamin, N., P.Dumas, J.Moncuit, W. H.Fridman, J. L.Teillaud, G. L.Carr, and G. P.Williams. 1998. Highly resolved chemical imaging of living cells by using synchrotron infrared microspectrometry.Proceedings of the National Academy of Sciences USA95:4837–40.
Martin, M. C., N. M.Tsvetkova, J. H.Crowe, and W. R.McKinney. 2001. Negligible sample heating from synchrotron infrared beam. Applied Spectroscopy55:111–13
Martoglio Smith, P. A.2000. Infrared microspectroscopy mapping studies of packaging materials: Experiment design and data profiling considerations.Vibrational Spectroscopy24:47–62.
Miller, L. M., and T. J.Tague. 2002. Development and biomedical applications of fluorescence-assisted synchrotron infrared micro-spectroscopy.Vibrational Spectroscopy28:159–65.
Roy, P., J.-B.Brubach, P.Calvani, G.deMarzi, A.Filabozzi, A.Gerschel, P.Giura, S.Lupi, O.Marcouill�, A.Mermet, A.Nucara, J.Orphal, A.Paolone, and M.Vervloet. 2001. Infrared synchrotron radiation: From the production to the spectroscopic and microscopic applications. Nuclear Instruments and Methods in Physics Research Section A467–68:42–36
Salvad�, N., T.Pradell, E.Pantos, M. Z.Papiz, J.Molera, M.Seco, and M.Vendrell-Saz. 2002. Identification of copper-based green pigments in Jaume Huguet's Gothic altarpieces by Fourier transform infrared microspectroscopy and synchrotron radiation x-ray diffraction.Journal of Synchrotron Radiation9:215–22.
Smith, G. D., L.Burgio, S.Firth, and R. J. H.Clark. 2001. Laser-induced degradation of lead pigments with reference to Botticelli'sTrionfo d'Amore. Analytica Chimica Acta440:185–88
Smith, G. D., and R. J. H.Clark. 2001. Raman and FT-Raman microscopy in art history and conservation science.Reviews in Conservation2:92–106.
Sommer, A., L. G.Tisinger, C.Marcott, and G. M.Story. 2001. Attenuated total internal reflection infrared mapping microspectroscopy using an imaging microscope.Applied Spectroscopy55:252–56.
Veiga, J. P., and M. O.Figueiredo. 2002. Sixteenth century tubular glass beads: Non-destructive chemical characterization using synchrotron radiation XRF. X-Ray Spectrometry31:300–4
AUTHOR INFORMATION
GREGORY D. SMITH obtained an undergraduate education at Centre College of Kentucky in chemistry and anthropology and continued his studies in the sciences and archaeology at Duke University before earning his doctorate in chemistry. After holding postdoctoral positions at University College London and the National Synchrotron Light Source, he is currently the Samuel Golden Research Fellow at the National Gallery of Art in Washington, D.C., where his research interests include applications of chemistry and vibrational microspectroscopy to the analysis of artists' materials. Address: National Gallery of Art, Scientific Research Department, Washington, D.C. 20565; g-smith@nga.gov
Section Index |